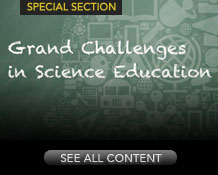
|
Special
issue of Science - 19 April 2013
Selection
of meaningfull articles related to teaching and learning science
in French context
Re-imaging science education and teacher professionnal development,
outside the pipeline for all students
Benoît
Urgelli
last
up-date : 04-Jan-2014 |
Introduction
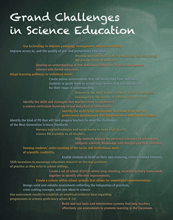
Plenty of Challenges for All
Pamela J. Hines,
Jeffrey Mervis,
Melissa Mccartney,
and Brad Wible
Science 19 April 2013:
290-291. |
if
students and parents around the world don't see the need for a
high-quality education in science, technology, engineering, and
mathematics (the so-called STEM fi elds), or mistakenly think
that they are already receiving satisfactory teaching in those
areas, then calls from the scientific community to improve STEM
education will fall on deaf ears.
we
explore the obstacles to progress, be they within the classroom,
across the school system, or in the larger social arena. We also
offer substantive suggestions on how to proceed.
Many
university faculty members are working to upgrade centuries-old
approaches to instruction. And, with a new emphasis on the practice
of science, promising assessment tools are being developed to
improve learning.
Yet
convincing the public of the importance of STEM education will
require more than explaining what the research shows or finding
ways to scale up best practices to reach the billions of students
who are entitled to a high-quality education. For scientists,
advances in science and technology arrive at such a rapid clip
that last years knowledge barely scratches the surface of what
is needed next year. At the same time, larger and more diverse
student populations clamor for access to knowledge. Not only will
the scientific workforce for the 21st century need skills and
knowledge we haven't even heard of yet, but all global citizens,
whether in their doctors office or in a polling booth, need to
be better informed. Turning the fire of the natural curiosity
of students into effective, flexible, and well-grounded outcomes
will take a concerted effort by many different actors. Among them,
scientists must play a central role. |
EDITORIAL:
Prioritizing Science Education
Bruce Alberts
Science 19 April 2013:
249. |
From
my many close contacts with outstanding U.S. teachers, I have
come to deeply appreciate their wisdom. They uniquely understand
today's 5- to 18-year-old students and have many valuable suggestions
for improving education systems. I am also painfully aware of
the many past failures that have been caused by not giving the
best teachers a strong voice in the public policies that profoundly
affect their profession.
More
generally, experience shows that actively soliciting advice from
those most intimately involved is essential for wise decision-making
at higher levels. Regrettably, education is one of the few parts
of U.S. society that fails to exploit this fact. Hence, my initial
Grand Challenge: Build education
systems that incorporate the advice of out-standing full-time
classroom teachers when formulating education policy.
A start has been made, but much more remains to be done (see the
Perspective by B. Berry on p. 309)
studies
reveal that the private sector seeks employees who can apply a
capacity for abstract, conceptual thinking to complex real-world
problems including problems that involve the use of scientific
and technical knowledgethat are nonstandard, full of ambiguities,
and have more than one right answer. These employees must also
have the capacity to function effectively in an environment in
which communication skills are vital in work groups.
Achieving the revolution in U.S.
science education that is called for in the Next Generation
Science Standards released last week would
go a long way toward creating the type of high-school graduates
that the private sector needs (see the Perspective by R.
Stephens and M. Richey on p. 313). Business leadership
in the United States often fails to advocate for wise education
policies, despite its potential for influence. Hence, my second
Grand Challenge: Harness the influence of business organizations
to strongly support the revolution in science education specifi
ed in the Next Generation Science Standards.
Rather than learning how to think
scientifically, students are generally being told about science
and asked to remember facts. This disturbing situation must be
corrected if science education is to have any hope of taking its
proper place as an essential part of the education of students
everywhere.
College science courses are taught by scientists, and they define
science education, modeling for teachers and adults what should
be done at lower levels. Most college faculty have not yet faced
up to the urgent need to improve on the standard one-size-fits-all
lecture format (see News story by J. Mervis
on p. 292). Thus, my final Grand Challenge: Incorporate
active science inquiry into all introductory college science classes.
|
Proficiency
(compétences)
in Science: Assessment Challenges and Opportunities
James W. Pellegrino
Science 19 April 2013: 320-323.
|
Proficiency
in science is being defined through performance expectations that
intertwine science practices, cross-cutting
concepts, and core content knowledge.
These descriptions of what it means to know and do science
pose challenges for assessment design and use, whether
at the classroom instructional level or the system level for monitoring
the progress of science education. There are systematic ways to
approach assessment development that can address design challenges,
as well as examples of the application of such principles in science
assessment. This Review considers challenges and opportunities
that exist for design and use of
assessments that can support science teaching and learning consistent
with a contemporary view of what it means to be proficient in
science.
Educational
assessments ought to be statements about what scientists, educators,
policy-makers, and parents want students to learn and become.
It is well established that what we
choose to assess will end up being the focus of instruction.
So, it is critical that science assessments, both external and
internal to the classroom, best represent the proficiencies we
desire.
Shared
Perspectives on Proficiency
A
disjuncture exists between students’ knowledge of science
facts and procedures, as assessed by typical achievement
tests, and their understanding of how
that knowledge can be applied through the practices of scientific
reasoning, argumentation, and inquiry (3, 4).
Seldom
has such a consistent message been sent as to the need for change
in what we expect students to know and be able to do in science,
how science should be taught, and how it should be assessed. The
emergent definition of proficiency is perhaps
most clearly expressed in three major elements of the U.S. National
Research Council (NRC) Framework for K-12 Science Education (1):
(i) core or “big” ideas within disciplinary areas,
(ii) practices of scientific and engineering reasoning, and (iii)
cross-cutting concepts. Collectively they define what it means
to know science, not as separate elements but as intertwined aspects
of knowledge and understanding [see also (12)].
These
statements move beyond vague terms
such as “know” and “understand” to more
specific statements like “analyze,” “compare,”
“explain,” “argue,” “represent,”
“predict,” “model,” etc. in which the
practices of science are wrapped around and integrated with core
content. Educators and researchers are also recognizing
that proficiency develops over time and increases in sophistication
and power as the product of coherent systems of curriculum, instruction,
and assessment.
The
virtue of such a view is that science educators are poised to
better define the outcomes desired
from their instructional efforts, which in turn
guides the forms of assessment that can help them
know whether their students are attaining the desired objectives,
as well as how they might better assist them along the way.
It is very important for the science education community, and
policy-makers and the public more broadly, to
develop a shared perspective on what constitutes high-quality
and valid science assessments across K-16+ if assessments are
to support teaching and learning and attainment of the desired
science education outcomes.
Proficiency,
Performance Expectations, and Assessment Design Challenges
The
Next Generation Science Standards (NGSS) (7) build
on these suggestions and include tables that define what each
practice might encompass and the expected uses of each cross-cutting
concept for students at each grade level.
This
is particularly important for the design of assessment materials
and resources that can be used in classrooms to support instruction
The
organization Achieve and its partners in NGSS development
have elaborated these guidelines into standards that are clarified
by descriptions of the ways in which students at each grade are
expected to apply both the practices and the cross-cutting concepts
and of the knowledge they are expected to have of the core ideas.
The NGSS appear as sets of performance
expectations related to a particular aspect of a core disciplinary
idea (see the draft example in Fig. 1). Each performance
expectation asks students to use a specific practice in the context
of a specific element of the disciplinary knowledge relevant to
the particular aspect of the core idea.
From
NRC Frameworks, Standards, and Performance Expectations to Assessments
The
process starts by defining the claims
that one wants to be able to make about student proficiency—the
ways in which students are supposed to know and understand some
particular aspect of a domain. Examples might include aspects
of force and motion or heat and temperature. The most critical
aspects of defining these are to be as precise as possible about
what matters and to express this in the form of verbs such as
“model,” “explain,” “predict,”
etc. In essence, the performance expectations found in the NGSS
are claims about student proficiency.
Science
Assessment Example Cases
Many
of the tasks that have been used for classroom assessment, and
those found in large-scale state, national, and international
tests, focus primarily on science
content or on aspects of scientific inquiry separate from content.
With few exceptions, such assessments
do not integrate core concepts and science practices
in the ways intended by the NRC Framework or NGSS.
Classroom
Instruction and Assessment
Several
of these projects (19,20,21,22,23) illustrate the feasibility
of designing tasks and situations, whether in paper-and-pencil
format or mediated via simulations embedded in technology, that
challenge students to reason with
and about core science concepts in life and physical science.
They demonstrate ways to obtain forms of evidence that can serve
multiple purposes, such as measurement of student proficiency
as well as diagnosis of student thinking for instructional improvement.
The SimScientists
(20,21) project has shown how assessment situations and tasks
involving dynamic simulations of science phenomena can be built
from a principled design process that supports classroom formative
assessment as well as summative assessment in large-scale state
programs (21).
National
and International Large-Scale Assessment
there are two large-scale assessment
programs that more closely exemplify aspects of
science proficiency that involve science practices: the U.S. National
Assessment of Educational Progress (NAEP) and the
Programme for International Student Assessment (PISA).
The
NAEP 2009 and 2011 assessments were constructed from a framework
document that identified specific areas of content in the life,
physical, and Earth and space sciences, as well as a set of science
practices: (i) identifying science principles, (ii) using science
principles, (iii) using scientific inquiry, and (iv) using technological
design. Item types fell into two broad categories: selected-response
items (such as multiple choice) and constructed-response items
(such as short answer). To further probe students’ abilities
to combine their understanding with the investigative skills that
reflect practices, a subset of the students completed hands-on
performance or interactive computer tasks (3, 4, 24, 25).
In
contrast to NAEP, which is administered to 4th-, 8th-, and 12th-
grade students, the PISA assessment
is administered only to 15-year-olds. The most
recent PISA science assessment results are based on a framework
that includes science proficiencies
that overlap with the science practices of the NRC Framework and
NGSS, as well as aspects of the NAEP framework
(26, 27).
Both
assessment programs are a source of examples of the types of performances
that align with the descriptions of proficiency discussed earlier.
[...] both might constitute reasonable
ways to monitor overall progress of science teaching and learning
in U.S. classrooms in ways consistent with implementation
of the NRC Framework and NGSS.
Advanced
Placement (AP) Science
The AP program offers college-level curricula to high school students.
Starting in 2006, the College Board, which administers AP, with
support from the U.S. National Science Foundation, initiated a
process that started by redefining the focus, critical content,
and science practices that should define proficiency at the end
of each AP science course (30). This would then guide development
of both a curriculum framework for each course as well as the
high-stakes assessment often used by colleges for purposes of
granting course credit and/or advanced course placement.
Using
the complementary processes of backward design (31) and ECD, a
framework was developed for each science discipline that is organized
in terms of disciplinary big ideas, enduring understandings, and
supporting knowledge as well as a set of seven science practices.
This structure parallels that of the core ideas and science practices
in the NRC Framework. Similar to what is advocated in the NRC
Framework and realized in the NGSS, performance
expectations or learning objectives were defined within each discipline
to reflect the blending of core ideas with science practices.
Through application of ECD, sets of claim-evidence pairs were
elaborated in each science discipline to focus and support course
instruction as well as development of assessment tasks for new
AP exams. [...] Figure
2 (Fig. 2 A sample of a short constructed response item
for the new AP biology exam.) provides an example of a short constructed
response item that involves the
integration of conceptual knowledge with aspects of the practices.
[...] AP
science instruction and assessment are changing in ways closely
aligned with the perspective on science proficiency described
earlier.
The
Road Ahead
Assessment
is a key element in the process of educational change and improvement.
Done well, it can signify what we want students to know and be
able to do and help educators create learning environments that
support attainment of those objectives. Done poorly, it sends
the wrong signals and skews teaching and learning. Our
greatest danger may be a rush to turn the NGSS into sets of assessment
tasks for use on high-stakes state accountability tests
before we have adequately engaged in research, development, and
validation of the range of tasks and tools needed to get the job
done properly. Most especially we
must ensure that teachers are given the time, support, and assessment
tools to create instructional environments where their students
have adequate opportunities to learn what is now expected of them.
|
Education
Forum Science Education Opportunities
and Challenges in Next Generation Standards
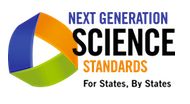
E. K. Stage,
H. Asturias,
T. Cheuk,
P. A. Daro,
and S. B. Hampton
Science 19 April 2013:
276-277.
|
understood
how human activity impacts Earth, including climate [...] learned
how to evaluate claims, argue from evidence, and understand models.
These understandings and practices are prominent in the U.S. National
Research Council (NRC) framework to guide the next iteration of
standards for U.S. elementary and secondary school students.
(Board on Science Education, National Research
Council (NRC), A Framework for K-12 Science Education: Practices,
Crosscutting Concepts, and Core Ideas (National Academies Press,
Washington, DC, 2011)). [...] this
effort will be more successful than previous attempts to use standards
to improve science education.
the
Next Generation Science Standards (NGSS)
are being developed by Achieve, a nonprofit organization, working
directly with 26 lead states.
Past
educational standards were developed by professional organizations
on behalf of scientists and educators and in different subject
areas independently, yielding more material than any K–12
school system (kindergarten to high school) could teach well (
6, 7). Now there is a call for “fewer, clearer, and higher”
standards. [...] skills and abilities
that support civic participation are explicit in the standards.
“The
ability to write logical arguments based on substantive claims,
sound reasoning, and relevant evidence is a cornerstone”
( 9). [...] Standards
for speaking and listening include “Integrate multiple sources
of information presented in diverse formats and media (e.g., visually,
quantitatively, orally) in order to make informed decisions and
solve problems, evaluating the credibility and accuracy of each
source and noting any discrepancies among the data”
( 3).
The
math standards take an overdue step toward greater
synergy with science by introducing modeling in secondary grades.
The math standards define modeling
as “the process of choosing and using appropriate mathematics
and statistics to analyze empirical situations, to understand
them better, and to improve decisions” (
4). The elaboration of the basic modeling cycle resonates with
the writing standards and with the science practices, e.g., “(5)
validating the conclusions by comparing them with the situation,
and then either improving the model or, if it is acceptable, (6)
reporting on the conclusions and the reasoning behind them. Choices,
assumptions, and approximations are present throughout this cycle”
( 4).
Standards
for Speaking and Listening
include, “Evaluate a speaker’s point of view,
reasoning, and use of evidence and rhetoric”
( 3). Standards for Mathematical Practice
include, “Construct viable arguments and
critique the reasoning of others” ( 4).
operationalized
“inquiry” with eight practices of
science and engineering: (i) asking questions and defining problems;
(ii) developing and using models; (iii) planning and carrying
out investigations; (iv) analyzing and interpreting data; (v)
using mathematics and computational thinking; (vi) constructing
explanations and designing solutions; (vii) engaging in argument
from evidence; and (viii) obtaining, evaluating, and communicating
information ( 2).
practices,
core disciplinary
ideas, and crosscutting concepts should not
be taught or assessed separately from each
other. Each draft science performance expectation
incorporates one or more disciplinary
idea, practice, and/or crosscutting concept.
Science
educators have decried the common practice of reading textbooks
instead of doing investigations; the former is still alive and
well ( 13). [...] It is time to embrace the coherence and learning
that can be achieved by making meaningful
connections between and among direct experience with science and
engineering practices and reading, writing, speaking, and listening
( 15).
Historically,
the United States has provided limited opportunity to learn science
to most of its students and advanced
training to a privileged few, focusing on the pipeline for future
scientists and innovators without concomitant attention to a science
literacy for citizenship. The system needs to
be transformed to affirm high standards of accomplishment for
all students and to provide resources for all students to reach
them ( 8).
some
states may reject the
NGSS because of the inclusion of evolution
and climate change ( 16). The National Center
for Science Education, a defender of teaching
evolution for more than three decades,
broadened its mission to include the defense
of teaching climate science.
Science
education benefits from the learning sciences; scientists
interested in the most effective teaching of science need to learn
from education research. Formal schooling has been criticized
as ineffective at motivating and inspiring students (17) and inadequate
at recognizing the relation between interest and accomplishment
(18). The NGSS can provide a platform
for formal education to become more motivating.
Many people are inspired by science in informal settings; parallel
attention to the NGSS can contribute to “a wide-ranging
and thriving ecosystem of opportunities that respond to the needs
of children as well as communities"(19).
Local
school districts, after-school providers, and informal science
institutions need to create a coherent strategy for the regional
science learning ecosystem.
|
Outside
the Pipeline: Reimagining Science Education for Nonscientists
Noah Weeth Feinstein,
Sue Allen,
and Edgar Jenkins
Science 19 April 2013: 314-317.
|
Educational
policy increasingly emphasizes knowledge and skills for the preprofessional
“science pipeline” rather than helping students use
science in daily life. We synthesize research on public engagement
with science to develop a research-based plan for cultivating
competent outsiders: nonscientists who can access and make sense
of science relevant to their lives. Schools
should help students access and interpret the science they need
in response to specific practical problems, judge the credibility
of scientific claims based on both evidence and institutional
cues, and cultivate deep amateur involvement in science.
For
half a century, the world’s wealthiest countries have asked
their education systems to teach science to all students, including
those who will not go on to scientific careers (1). Under slogans
such as “science literacy” and “science
for all,” schools have attempted to prepare all
students to make sense of science in daily life. With the exception
of modest and isolated gains in conceptual knowledge (2),
it is not clear that these campaigns have enhanced people’s
ability to function in a world where conflicting health advice
clutters the Internet, research is filtered through political
screens, and the media strips context from scientific claims.
These
results should provoke renewed interest in the relationship between
science education and public engagement with science and the pursuit
of more fruitful forms of science literacy. Instead, many scientists
and policy-makers are turning their attention away from the role
of science in daily life and advocating a greater focus on the
so-called “pipeline”:
preprofessional education that delivers science-ready students
to colleges and universities (3). Even crusaders
for science literacy take for granted that scientific training—of
the same sort that prepares students for scientific practice—will
help nonscientists navigate fields as diverse as personal health,
politics, the economy, leisure, and employment (1, 4, 5). There
is little empirical evidence to support this assumption. On the
other hand, a growing number of studies show untrained citizens
engaging with science in adaptive ways (6). These citizens, whom
Feinstein refers to as “competent outsiders” (7),
identify relevant pieces of science and understand their local
or personal implications without relying on school-based knowledge
of particular scientific methods or concepts (6, 8).
How
can education help more people act like competent outsiders?
competent
outsiders: nonscientists who can access and interpret
the science most relevant to their lives. We reconsider
established goals of science education in light of three
central findings about public engagement with science and discuss
implications for research and practice.
How
People Interact with Science
Research
shows that different groups interpret science differently (6,
9–12). An Alzheimer’s advocacy group, biotech investment
firm, and religious coalition may all be interested in stem cell
research, but different motivations
underlie their interest and shape their engagement.
Social, cultural, and demographic differences influence how people
engage with science, both in school (13) and out (6, 11).
For example, communications researchers have identified six demographically
distinct groups of Americans who respond to news about climate
change in predictable, group-specific ways (11). Local
knowledge and experience, such as the history of tension between
rural residents and a nuclear power plant (14), can play an important
role. There are
many different “publics” for science, each with different
concerns and resources for making sense of the world.
To
complicate matters, science is not a single, uniform thing. Science
education places particular value on experimentation, but some
fields rely on observational data or simulations, whereas others
are devoted to theoretical inquiry. Even closely
related fields can diverge on important matters, such as the validity
of research methods or the nature of acceptable evidence (15).
Nonscientists typically interact with specific manifestations
of science rather than “science” as a whole (6, 12,
16). Although scientists may agree on abstract principles (such
as hypothesis-testing) and methodological heuristics (such as
model-building), the science of climate modeling is very different
from the science of clinical trials, and understanding the family
resemblance between them may not help a layperson make sense of
evidence.
This
leads to perhaps the most important finding: Although
some people are interested in science for its own sake, many engage
with science in response to situation-specific needs and tend
to be interested in science only insofar as it helps them solve
their problems (6–10, 12). Thus, a mother
seeking therapies for her autistic son may explore research literature,
but she is not attempting to understand that literature from a
scientist’s perspective. Instead, she labors to integrate
what she learns with her knowledge of local services and her first-hand
understanding of her child (16). Context
shapes the process of engagement, and scientific principles take
on different significance in different contexts, where they are
laden with social and ethical implications (8,
10, 12). [...] Scientific
understanding may contribute to the solution, but will rarely
be the entire solution. It is important to be
realistic about the sort of understanding people seek—and
need—to make decisions (17).
Reconsidering
the Goals of Science Education
These
findings about public engagement with science strain the credibility
of established approaches to science education. Scientists, educators,
and policy-makers claim that science education is useful (1–3,
5), but what use is it to know a canonical collection
of facts or an allegedly generic scientific method if people engage
with specific pieces of science in highly contextualized ways?
Can education prepare students for the deep idiosyncrasy of daily
life? Evidence on public engagement indicates that students should
still know science, think scientifically, and appreciate science—but
it may be necessary to reconsider the established interpretation
of these goals and the strategies used to achieve them.
1.
Knowing Science: From Knowing the Textbook to Accessing the Science
You Need
No
set of scientific concepts and principles, no matter how carefully
chosen, will be sufficient preparation for future engagement with
science. This is a consequence of the unpredictable path
of scientific progress, shifting social and political
demands on scientific knowledge, and the variety of contexts and
motives that drive public engagement. Even if it were possible
to predict the future of science, one could never anticipate how
science will ripple through the diverse future lives of students
(4, 9, 12). Yet prior knowledge is only one piece of the sense-making
apparatus that people use in their encounters with science. When
reading a scientific article, a person draws on prior knowledge
to interpret the text, but she does not stop when she is unfamiliar
with a concept or uncertain of implications; she looks up the
concept online, cross-references a second article, discusses the
matter with friends, and seeks out complementary expertise (6,
16, 18). People employ social and material resources to
solve problems and answer questions, and encounters with science
are an impetus for new learning as well as tests of prior knowledge.
Resources
alone do not guarantee fruitful engagement with science: The same
literature that reveals impressive sense-making ability among
laypeople also reveals failures, frustrations, and uneven competence
(6, 12, 16). Science education should prepare more students
to access and interpret scientific knowledge at the time and in
the context of need. Public engagement with science is
not simply the application of scientific knowledge;
it requires translating a daily problem into scientific
terms and reconstructing the scientific answer amid the constraints
of daily life (6, 12, 16). A rural resident worried
about pesticide contamination must learn to express his concerns
in questions that science can answer: What pesticides, at what
doses, are most harmful? Are there reliable tests for pesticides
in my children’s air or water? These questions lead to answers
that must then be translated back into local reality: Who will
help me test my water? What can I do to mitigate the risks? The
decision-making process incorporates both scientific and nonscientific
information.
One
promising approach for preparing students to succeed in such circumstances
is Problem-Based Learning (PBL),
which confronts students with ill-structured challenges, asking
them to extend their existing knowledge and develop concrete solutions
(19). PBL can produce durable knowledge gains and foster metacognitive
skills that underlie self-directed learning, although researchers
have yet to identify which features of PBL contribute most to
learning (19, 20). Developed in medical schools,
PBL needs further validation in kindergarten through grade 12
(K-12) settings, but it shares features with other
promising pedagogies specific to K-12, such as Science-Technology-Society
(STS) and Place-Based Education (21, 22). All
of these mimic public engagement with science by making the problem
a focus for learning, allowing students
to develop complex questions and test the adequacy of their answers,
and, in many cases, using authentic social and practical problems
that cannot be defined in purely scientific terms.
Fundamental
problems of research and practice must be addressed before these
pedagogies can be used to greatest effect. Little is known about
using them together, or over time, to help students recognize
when and how science is relevant. Students frequently struggle
to apply what they have learned in one specific context to another;
on the other hand, teaching generic problem-solving skills appears
to have limited value (23, 24). Finding the right level of specificity,
and honing strategies to connect multiple learning episodes, are
problems of longstanding interest to researchers and educators
(23, 25). Educators and researchers
should work together to adapt problem-focused pedagogies for a
broad range of audiences, develop appropriate assessments, and—critically—find
the most productive balance between these strategies and other
means of presenting disciplinary science content.
2.
Thinking Scientifically: From Practicing Science to Judging Scientific
Claims
“Thinking
scientifically” has been interpreted in many ways,
from the trial-and-error experimentalism of early
progressives to the scientific method dogma of
the post-war era and the more flexible (if also more vague) idealism
of scientific inquiry
(1, 5). In the United States, the
forthcoming Next Generation Science Standards decompose scientific
inquiry into distinct but interconnected “scientific practices”
such as modeling, argumentation from evidence, and communication
of results. This is an important step
forward. It rejects the empirically dubious notion of a single
scientific method, offers greater specificity than most inquiry
frameworks, and better represents the collaborative and iterative
aspects of scientific work (5).
Yet
the scientific practices approach still emphasizes the scientist’s
“insider” perspective, neglecting cues that help outsiders
make informed judgments. Nonscientists rarely need to replicate
the iterative processes of systematic research, and literature
suggests that it is difficult to transfer principles of
research design, learned in disciplinary contexts, to the highly
variable circumstances of daily life (23, 24). On the
other hand, nonscientists do need to judge the trustworthiness
and local validity of putatively scientific claims. Studies
show that competent outsiders make sophisticated judgments about
the credibility of scientific claims based on cues like professional
reputation, publication venue, institutional affiliation, and
potential conflicts of interest, even when they do not understand
technical nuances of experimental design or laboratory technique
(6, 8, 10). In one classic sociological study, local knowledge
and historical context, combined with direct observation of scientists
in the field, helped farmers make sophisticated counterarguments
to government-sponsored studies when their grazing lands were
contaminated by radioactive fallout (14). Studies
have emphasized the importance of trust, reputational networks,
and heuristic reasoning in judgment and decision-making
(10, 26).
Science
education could do far more to help people judge scientific claims
based on the information available to them. This is important
given the decline in dedicated science journalism at for-profit
news organizations; increasingly, citizens are turning to the
Internet, with its variable quality and political motivations,
for science news (10). Lessons that
focus on scientific argumentation and communication are part of
the solution because they help students understand how scientists
evaluate evidence and how research is packaged for presentation
to various audiences (5, 27). Yet even this shortchanges
the histories, institutions, and norms that contribute to the
reliability of scientific knowledge. Competent outsiders
appreciate the socio-political nuances of
“how science really works,” including scientific credentials,
the role of peer review in research funding and publication, and
the differing perspectives of the many types of research organizations
(8, 10). They can navigate the changing world of popular
science media, recognizing signs of source bias and understanding
the difference between journalistic and scientific accounts of
research (10).
This
material can be dry and inaccessible when presented out of context,
but promising pedagogies offer platforms
for examining scientific credibility in realistic contexts.
In Socio-Scientific Issue Discussions (SSID), students engage
in structured conversation about a science-inflected social problem,
with the goal of uncovering epistemic and ethical nuances at the
interface of science and daily life (28). Other
strategies focus on the creation and interpretation of science
texts, ranging from research articles to popular science journalism
(29, 30). These pedagogies must be refined to reveal the social
and institutional structures of science. Although both address
the credibility and usefulness of different sources, and both
provide apt venues for exploring issues of institutional trust,
work is needed to develop a systematic and developmentally appropriate
set of scaffolds for learning about
topics such as peer review and conflicts of interest.
3.
Appreciating Science: From Positive Feelings to Deep and Durable
Involvement
Most
adults in high-income countries express mild but consistent interest
in scientific topics (31), but formal education may have little
to do with this: A substantial fraction
of students in those same countries lose interest in science as
they progress through school (32). Schools may
lag behind informal learning environments in their ability to
inspire and develop students’ interest in science (33).
Older, top-down mechanisms for public engagement are being joined
by science cafes, participatory science games, and maker spaces
(community-oriented places that foster collaboration and resource-sharing
in small-scale design and fabrication projects). Children and
adults may connect with science through “citizen science”
and “professional-amateur” communities dedicated to
phenology, astronomy, and even molecular biology (18). People
who interact with science through these platforms do so for widely
varying reasons connected to personal interest and social identity
(18, 33). In this rich and dynamic context, how and why
should schools continue to foster appreciation of science?
Research
suggests that deep, personal interest in some field of science
provides motivation for future interactions, even with science
in unrelated fields. Students who pursue their own science-related
interests have a stronger sense of their ability to learn science
in the future (33) and are less likely to lose interest over time
(34). Their involvement in personally
or socially meaningful science-related activities can lead to
learning experiences that resemble project-based learning and
socio-scientific issue discussions (35). When
students find a particular scientific topic compelling, they seek
experiences that prepare them for future encounters with science.
Knowledgeable amateurs can become powerful resources for their
communities (9, 18, 33).
Schools
wishing to develop deep and durable involvement in science
should embrace the diversity of student interests—a
challenge for educational systems accustomed to pushing everyone
toward the same goal. Three pathways hold promise. First,
educators can use the flexibility
provided by project- and place-based pedagogies to help students
identify and develop individual interests and expertise.
Second, schools can pursue partnerships
with museums, which excel at sparking curiosity,
and with afterschool clubs and community organizations, which
provide flexible spaces for ongoing exploration (33). Third, educators
can integrate science-based games and citizen science engines
like FoldIt and GalaxyZoo into their curricula. Researchers should
develop efficient ways to track the development of lasting student
interests and identify productive ways to integrate informal experiences
and game-based technologies into schools and classrooms (33).
Implications
On
the way to becoming competent outsiders, students should
learn to (i) access and interpret science in the context of complex,
real-world problems; (ii) judge the credibility of scientific
claims based on both social and epistemic cues; and (iii) cultivate
deep and durable involvement in science, even when it takes them
away from the formal curriculum. In practice, this means moving
strategies such as PBL, SSID, and interest-driven student exploration
from the pedagogical margins to the center.
Allotting more time and resources to these strategies will result
in a better balance between preprofessional science education
and science education for nonscientists; given
that PBL is used in a range of academically rigorous contexts,
it may pay dividends for future scientists as well.
These
strategies are works in progress. Too few studies
investigate the challenges of moving from practical problems to
scientific questions and integrating science back into practical
solutions. Too few studies identify skills needed to reverse-engineer
a robust and coherent knowledge structure using real-world resources.
Educational research on scientific epistemology neglects the diverse
circumstances in which people encounter scientific claims, as
well as the social and institutional knowledge that contributes
to evaluating those claims. Research on deep and durable involvement
in science is in its infancy; although there are portraits of
success in games-based learning and informal science education,
practice outstrips research. There
is an urgent need to understand how and why these settings succeed
(and fail) to transform attitudes, motivation, and identities.
Educators
should not wait for these questions to be answered.
Useful research requires real-world cases to study,
and it is educators who will do much of the work of adapting project-based
learning and other strategies to diverse K-12 settings.
Predictable challenges loom: School schedules, parent expectations,
and high-stakes testing militate against pedagogies that sacrifice
short-term knowledge gains for complex skills, increased motivation,
and a narrower but longer-lasting body of knowledge. Teachers
and administrators should work together to clear space for pilot
programs that test and demonstrate the value of these approaches.
It may be most effective to deploy them as solutions to other
widely acknowledged problems. For example, STS education produces
motivational gains among students who are less likely to enroll
in science courses (21), whereas PBL has found early champions
in gifted education, with students who may have exhausted their
local course offerings (19). Pilot programs conducted in these
contexts can serve as beachheads for broader adoption.
Scientists
may be allies or adversaries in reform. Some have played
a decisive role in pedagogical and curricular progress, whereas
others have defended the battlements for the established facts-and-principles
approach (1). The scientific
pipeline dominates educational discourse today,
but it is those outside the pipeline who would benefit most from
reform. Serving their needs requires
a different sort of activism, and new attention to evidence about
how, when, and why people interact with science.
|
Physical and Virtual Laboratories
in Science and Engineering Education
Ton de Jong,
Marcia C. Linn,
and Zacharias C. Zacharia
Science 19 April 2013:
305-308. |
The
world needs young people who are skillful in and enthusiastic
about science and who view science as their future career field.
Ensuring that we will have such young people requires initiatives
that engage students in interesting and motivating science experiences.
Today, students can investigate scientific phenomena using
the tools, data collection techniques, models, and theories of
science in physical laboratories that support interactions with
the material world or in virtual laboratories that take advantage
of simulations. Here, we review a selection of the literature
to contrast the value of physical
and virtual investigations and to offer recommendations for combining
the two to strengthen science learning.
Policy-makers
worldwide recommend including scientific investigations in courses
for students of all ages (1, 2). Research
shows advantages for science inquiry learning where students conduct
investigations compared with typical instruction featuring lectures
or teacher demonstrations (3, 4). Investigations
provide opportunities for students to interact directly with the
material world using the tools, data collection techniques, models,
and theories of science (1). Physical, hands-on investigations
typically fill this need, but computer technologies now offer
virtual laboratories where investigations involve simulated material
and apparatus. The value of physical
laboratories for science learning is generally recognized (1),
but the value of virtual, simulated alternatives for hands-on
physical laboratories is contested (5). We explore
whether this hesitation concerning virtual laboratories is justified.
Empirical
Studies Comparing Physical and Virtual Laboratories
These
studies show advantages for each type of laboratory, as well as
trade-offs. Benefits of virtual
laboratories arise when students can investigate unobservable
phenomena that are not found in the physical investigation,
conduct many more experiments than are possible in the physical
setting, link observable and atomic level phenomena, or contrast
different depictions of similar phenomena. Physical
laboratories have advantages when the instructional goal is to
have students acquire a sophisticated epistemology of science,
including the ability to make sense of imperfect measurements
and to acquire practical skills.
Combining
Physical and Virtual Laboratories
Students in the combined condition outperformed those in the physical
alone and virtual alone conditions, attesting the value of the
combination over both other conditions.[...] Overall, well-designed
combinations of virtual and physical experiments compared with
either one alone allow students to gain a more nuanced understanding
of scientific phenomena and a more robust understanding of inquiry.
|
Professional
Development for Science Teachers
Suzanne M. Wilson
Science 19 April 2013: 310-313.
|
The
Next Generation Science Standards will require
large-scale professional development (PD) for all science teachers.
Existing research on effective teacher PD suggests factors that
are associated with substantial changes in teacher knowledge
and practice, as well as students’ science achievement.
But the complexity of the U.S. educational system continues to
thwart the search for a straightforward answer to the question
of how to support teachers. Interventions that take a systemic
approach to reform hold promise for improving PD effectiveness.
Traditionally,
much PD has focused on enriching teachers’ content knowledge
(CK), introducing
new curriculum and instructional materials, enhancing pedagogical
CK, or educating them about scientific inquiry. [...] only
PD that involved teachers examining
student thinking and considering the implications for instruction
was associated with increases in both teacher and student science
knowledge. [...]
In
the Science
Teachers Learning through Lesson Analysis (STeLLA) project,
teachers participated in PD that involved analyzing science teaching
practice using video cases (16). [...] The
program substantially improved teachers’ CK and their ability
to analyze science teaching. Students of STeLLA teachers also
demonstrated considerably higher gains in their science CK.
researchers lack a clear
theory of the underlying mechanisms involved in teacher learning.
For instance, researchers have argued that teachers’ increased
CK leads to better self-efficacy (19). In turn, this increased
efficacy leads to higher levels of persistence. Thus, teachers
who increase their CK also improve their confidence, which leads
to more motivation and perseverance as teachers learn to educate
in fundamentally different ways. The five general characteristics
listed above—duration, active learning, collective participation,
coherence, and content focus—are design features, but future
research will need to explore how these features work together
to produce teacher learning. Using the above example, does a focus
on content lead to greater teacher confidence, which, in turn,
leads to greater active engagement and, eventually, higher student
achievement?
A
more complex view of teacher learning is clearly needed, one in
which professional learning is seen as more dynamic and iterative,
connecting teachers’ experiences in their classrooms with
formal opportunities for collective reflection and for acquiring
new knowledge that targets genuine problems of practice (17).
Models of teacher learning should also account for the internal
coherence of a school’s leadership, culture, curriculum,
assessments, and PD, as teachers learn inside of organizations
that fundamentally shape their interests in and abilities to learn
from practice (20).
PD
Embedded in School Reform
In a longitudinal study of school change, one
research team identified five supports for change:
(i) leadership (principals who are strategic, focused on instruction,
and inclusive), (ii) professional capacity (teacher quality, their
beliefs about change, their capacity to work collaboratively,
and the quality of ongoing professional development), (iii) parent-community
ties (schools that are welcoming to parents and have strong connections
to local institutions), (iv) student-centered learning environments
(schools that are safe, nurturing, stimulating, and welcoming),
and (v) instructional guidance (the organization of the curriculum,
its academic rigor, and the tools teachers have to advance learning)
(21). The researchers found that the real value of these supports
was in their combined strength: Schools with strength in three
to five of these supports were 10 times more likely to demonstrate
significant learning gains (as measured in mathematics and reading).
Initiatives that take a systemic
view of educational improvement present challenges to those who
want to draw causal conclusions about the contributions of specific
components of the reform to student learning. Teachers’
knowledge and perceptions, administrative support, PD, and available
resources, among other factors, are intertwined with student experiences
with inquiry, teachers’ collaborations, technology use,
and other aspects of the system.
It is nearly impossible to isolate the effects of PD on student
learning.
Pressing
Challenges
The
NGSS present a view of science teaching that differs
from the standard fare in U.S. classrooms. Getting from here to
there will require considerable investment of resources: The research
described here required considerable development of highly specified
instructional materials and tools to support teachers and students
in using those materials; additionally, this research validated
ongoing assessments of learning and responsive, extensive PD.
Responding to the NGSS will require the development of many more
such resources, and this innovation will need a coordinated system
of research to empirically document the hallmarks of effective
instruction, the qualities of effective materials, and the dynamics
of high-quality PD. Although the
array of available PD in this country is extraordinary, we cannot
afford such broad experimentation without learning from it so
that we can much better align the resources spent on PD
(estimates range from $1 billion to $4 billion per year) with
the demands teachers face in today’s classrooms.
Recent
research has begun to explore this challenge by offering PD that
integrates learning science and literacy. One
such intervention focused on promoting the learning of science
inquiry by students from linguistically and culturally diverse
backgrounds (23 : Improving science
inquiry with elementary students of diverse backgrounds -Cuevas,
P (Cuevas, P); Lee, O (Lee, O); Hart, J (Hart, J); Deaktor, R
(Deaktor, R) -JOURNAL OF RESEARCH IN SCIENCE TEACHING Volume:
42 Issue: 3 Pages: 337-357). [...] Students
who participated in the treatment classrooms demonstrated significantly
higher achievement on district benchmark tests in science and
reading, as well as on the state reading exam, but not on state
science tests.
Online
PD has the potential for providing “just-in-time assistance”
and is potentially more scalable than PD that presses on limited
local resources. In addition to online courses, other emerging
environments—such as multi-user virtual environments in
which the participants take on avatars in virtual worlds, augmented
realities in which participants in their own real-world contexts
interact with a virtual setting, and social networks that connect
teachers across the country—also hold promise for increasing
teachers’ access to relevant, high-quality science PD and
materials (26). To date, not enough research exists to help us
understand the affordances and limits of these venues.
we
need a stronger theoretical base
that reflects the complex ecology in which teachers work and learn.
[...] education reform that leads
to fundamental change, such as that envisioned in the NGSS, requires
time [it takes several years for teachers to change
their practice (6, 27, 28)]. Reform efforts also require investments
in infrastructure (leadership, teacher networks, planning time),
the organizational coherence that encourages teachers to take
risks and learn new content, parents to support the new standards,
and students to demonstrate the perseverance and curiosity needed
to achieve scientific literacy.
|
Teacherpreneurs:
A Bold Brand of Teacher Leadership for 21st-Century Teaching and
Learning
Barnett Berry
Science 19 April 2013: 309-310.
|
Challenges
facing our public schools demand a bold brand of teacher leadership.
Teacherpreneurs, effective teachers who teach students regularly
but also incubate and execute the kinds of policies and pedagogies
students deserve, represent a new
culture of training and ingenuity. Teachers who
lead outside the classroom but do not lose their connection to
students are best positioned to develop and disseminate
best policies and practices for 21st-century
teaching and learning.
Since
the release of the Coleman Report in 1966, there has been a steady
drip of empirical evidence showing that teachers are the
most crucial in-school factor for student learning (1–4).
And while U.S. policy-makers have sought to reform education by
improving teacher quality, in doing so they have not paid a great
deal of attention to the research on teaching and learning. Making
matters more complicated, America’s
approach to the teaching profession has been driven more
by ideological agendas and power politics than by scientific evidence.
[...] many reformers fail to envision schools that look different
than they did when they were students. We have entered a new era
with advanced learning technologies (5) and growing scientific
evidence on how humans learn, with enormous implications for teachers
and teaching (6). New methods of assessing cognition, emotion,
and learning make it possible for teachers, if they are well prepared
and supported, to serve students in ways previously unimaginable
(7, 8). [...] Although teachers are paramount to student learning,
too few education policies promote
leadership from those who teach.
Why
We Need a Bold New Brand of Teacher Leadership
Isolated
behind the closed doors of individual classrooms,
teachers traditionally have had little time to observe and learn
from their peers.
However, education research demonstrates
that when teachers collaborate with one another, their students’
achievement improves (9). Economists, using sophisticated
statistical methods and large databases, have concluded that students
score higher on achievement tests when their teachers have opportunities
to work with colleagues over long periods of time and spread their
expertise
(10). And in the 2009
MetLife Survey of the American Teacher, over 90% reported
that their colleagues contribute to their individual teaching
effectiveness (11).
Richard
Elmore made the compelling case that many education policies and
practices often wither, primarily because reformers fail to “develop
organizational structures that intensify and focus” the
new reforms supported by too few “intentional processes
for [the] reproduction of successes (12).” His research
pointed out that reform is about learning, and for teachers to
teach more effectively they must have “encouragement and
support, access to special knowledge, time to focus on the requirements
of the new task, time to observe others doing it (12).
[...] But
these conditions rarely are in place. Compared to teachers
in nations with top-performing education systems, like Singapore,
most U.S. teachers have very limited access to leadership and
learning opportunities or time to engage in high-quality professional
development—such as the “lesson
study” common in Singapore and Shanghai (13).
Because of the hierarchical structure of U.S. schools,
teachers who want to lead outside their own classroom have had
to leave it to become administrators, district leaders, or policy-makers.
The
Coming Age of Teacherpreneurs
America’s
public education system needs teacherpreneurs—classroom
experts who teach students regularly, but also have time, space,
and reward to spread their ideas and practices to colleagues as
well as administrators, policy-makers, parents, and community
leaders. The Center for Teaching Quality has supported
as well as documented how this special brand of teacher leaders
has begun to serve as online coaches, edugame developers, community
organizers, and policy analysts, without leaving the classroom
(14). In doing so, they have begun to solve problems of student
and teacher learning that today’s reformers have yet to
identify. Daunting barriers remain,
including the relatively large number of educators in school systems
who never teach, the highly prescriptive teaching day, and top-down
reformers whose political agendas are out of sync with the ideas
of classroom experts. However, teacherpreneurs, because of their
deep knowledge of students, families, and communities, are more
likely to be embraced by their colleagues.
I
am optimistic. Most Americans have
trust and confidence in individual teachers (15),
and new technologies that amplify teachers’ collective wisdom
and the impact of their leadership will resonate with parents
and the public. Additionally, MetLife’s
most recent survey revealed that one in four teachers
nationwide are extremely or very interested in hybrid roles that
would allow them to both teach and lead outside their schools,
districts, and states (16).
While
these classroom experts should be highly paid,
teacherpreneurship is not mainly about establishing a new income
stream for underpaid professionals. It is much
more about rewarding a new culture
of schooling and creativity. As Peter Drucker
said of entrepreneurs almost 50 years ago, “search for change,
respond to it and exploit opportunities (17).” It
is time for America to cultivate teacherpreneurs who will do the
same, deepening and spreading best policies and practices for
21st-century teaching and learning.
|
Generating
Improvement Through Research and Development in Education Systems
M. Suzanne Donovan
Science 19 April 2013: 317-319.
|
To
effectively address problems in education, research
must be shaped around a problem of practice. Reorienting
research and development in this way must overcome three obstacles.
First, the incentive system for university researchers must be
changed to reward research on problems of practice. Second, the
contexts must be created that will allow the complexity of problems
of practice to be understood and addressed by interdisciplinary
teams of researchers, practitioners, and education designers.
And third, meaningful experimentation
must become acceptable in school systems
in order to develop a better understanding of how to effectively
stimulate and support the desired changes.
When
research informs designs that solve a problem from the point of
view of the users, barriers to change disintegrate (4). [...]
when an innovation requires that people change their behavior
to achieve goals others have set—[...] to motivate
teachers to engage students in classroom discourse rather than
to teach through lectures (6)—it is an implementation
challenge. [...] Research and development (R&D) will therefore
need to address both design and implementation challenges.
Identifying
the Right Problem
Scientific
research can be driven either by theory or by problems of practice.
[...] The National Institutes of Health and the National Science
Foundation support programs of “translational research”
intended to make advances in research knowledge usable for practice
[...] It needs only to be put into the language of practice.
Rarely
do problems of education practitioners map neatly onto areas of
scientific research [...] Pasteur’s
scientific breakthrough came with a commission to work on a practical
problem: the spoiling of wine (11). The problem-solving
research did not end with the realization that bacteria cause
the spoiling, nor with the evidence that heat could be used to
destroy bacteria. The heating process changes the end product—whether
wine or milk—affecting taste, appearance, and digestibility
(12). It took decades of work on the time and temperature of heating
and cooling to develop the process of pasteurization that revolutionized
the delivery of milk (13). The translation
metaphor conceals the way in which research that solves problems
of practice is shaped and disciplined by the problem to produce
usable and desirable solutions.
A
National Research Council Committee that explored the weak relationship
between research and practice in education (1) concluded that,
in contrast to medicine and agriculture, education researchers
have few opportunities to identify the specific problems of practice
that can serve as productive starting points for programs of research
and development. For example,
research illuminates the influence of social and emotional factors
on learning (14).
If students think effort matters, they are more likely to persevere
when schoolwork becomes difficult than if they believe intelligence
is fixed (15), and they will be exposed as having too little.
And if a student believes he or she is expected to do poorly—a
condition that can be manipulated in experiments—performance
declines (16).
The importance of these findings is clear, but how they might
be effectively incorporated into practice is not.
[...] One
opportunity for intervention in the classroom arises when students
give incorrect answers (17). At that moment a teacher can further
explore the student’s thinking, signaling both the expectation
that struggling will produce learning, and that the student is
capable of thinking further about the problem. However,
when teachers call on another student or provide the answer themselves,
their responses are consistent with a belief that “some
people have it and some people don’t.”
Finding
Effective Solutions
Identifying
the obstacles to full implementation resulted in a list of 30.
Patient behaviors on the list, familiar to any educator,
include defiance, lack of knowledge, immortality complex, inadequate
parental support, and absence of consequences. Other obstacles
shared with education systems include lack of coordination among
professionals, legitimate activities of lesser importance, inadequate
staffing, and supply shortages.
[...] A
multidisciplinary team tackled high-leverage component problems.
Psychologists used well-researched approaches for changing the
behavior of adolescents, including the introduction of signed
contracts, performance monitoring, and providing small rewards
for follow-through.
The
initial identification of “best practice” is only
a starting point for improvement. Designing a
sustainable solution required varied expertise and inputs from
medical practitioners, psychologists, administrators, and supply
managers. And leadership was needed to galvanize the cross-functional
teams toward a common purpose (21). A second grand challenge thus
emerges: Can we create the settings
in education where multidisciplinary researchers, education designers,
and education practitioners are led and supported to follow the
contours of problems in order to identify systemically sustainable
solutions?
Getting
Solutions to Spread
Sociologists
look for answers in the culture and social norms of organizations.
People are more likely to do what others around them do, as evidenced
in smoking, eating, and exercising habits (26). In
education settings, social norms that reject changes or prohibit
questioning of a professional’s practice are impediments
to change (19, 27).
[...] Psychologists
look for answers in the individual’s cognitive and emotional
processes (28). External incentives and organizational norms are
part of an individual’s calculus, but influences also include
personal goals, orientations, knowledge, and resources (29), as
well as expectations of outcomes based on beliefs about one’s
ability to succeed and about reactions from others (30). Control
over the conditions for behavior change (31), and the specificity
of an individual’s planning, also matter (32).
28
: A. H. Schoenfeld, Toward professional development for teachers
grounded in a theory of decision making. ZDM 43, 457 (2011).
31 : D. J. Terry, J. E. O'Leary, The
theory of planned behaviour: The effects of perceived
behavioral control and self-efficacy. Br. J. Soc. Psychol. 34,
199 (1995).
In
a comprehensive study of psychological
theories of behavior change, researchers in the
field of “implementation science” have attempted to
understand which theoretical explanation for individual behavior
has the greatest predictive value with regard to medical practitioners’
decisions (33). Several of the theories,
as measured in the study, had no predictive value.
Despite the breadth of the variables included, the best-performing
theories explained between 25 and 42.6% of the variance in intended
behavior but only 2.4 to 6.3% of actual behavior (33).
what
can be done? Invest further in the scholarly endeavor of understanding
professional behavior, as implementation scientists are attempting?
Deepen the knowledge base on how education organizations use research
knowledge, and how they might do so more effectively? Engage in
problem-solving research and development, and learn about human
and organizational behavior in the process of experimenting with
changing it (24)? All would no doubt be valuable. But
if the ultimate goal is to improve practice, then problem-solving
R&D has two features to recommend it.
First, the lag time between the initial investment in research
and change in practice will be shorter if the new knowledge is
generated as a by-product of testing interventions in practice.
This is particularly important if practitioners are to see value
in research. [...] when both the importance and
the variety of contexts are high, as with shifts in professional
practice, generating knowledge by intervening and observing what
happens is likely to be more illuminating. If, for example, teachers
shift more willingly to inquiry teaching when they have routine
opportunities to problem-solve with their colleagues, when the
materials are replenished by a designated staff member, and when
parents are given weekly updates about science activities, it
is difficult to imagine that such contextual factors could be
identified without experimenting in practice settings.
Moving from practice to theory is likely to be more fruitful than
the reverse. But it will require that meaningful experimentation
in education settings become acceptable. This is the third of
the grand challenges.
Supporting
Experimentation in Education Settings
The
concept of experimentation does not sit well in systems that are
highly accountable to the public. Parents would
need to be persuaded that their children’s education was
not being jeopardized. And school system administrators and teachers
who have many constituencies, none of which clamor for experimentation,
would need to have the time, resources, flexibility, and incentives
to engage in experimentation.
[...]
If, for example, there were practice sites where
R&D collaborations were supported routinely (challenge 2),
then researchers may find time spent working in those settings
to be more productive and more supportive of goals related to
quality and number of publications (challenge 1). And consistent
financial support for such sites would provide an incentive to
overcome an aversion among educators to experimentation, allow
for the development of a different culture that is consistent
with the designation as an R&D site, and provide the potential
for a shift in the perception of parents regarding experimentation
(challenge 3)—just as hospitals associated
with experimental research and development are often sought out
because they are on the leading edge.
There
are signs of movement. [...]. [...] There will be no “silver
bullets” that will transform education systems from the
outside—not in the form of new standards, assessments, programs,
or technologies. While these changes are important, they will
come up against inevitable implementation barriers. But
if we create the organizational capacity for researchers and design
experts to work with practitioners inside the system, we could
potentially change the outcome [...]
|
|